Gustavo Matute-Bello (1) and Michael A. Matthay (2) From the (1) Pulmonary & Critical Care Division, Center for Lung Biology, University of Washington, Seattle, WA and (2) the Cardiovascular Research Institute, University of California, San Francisco, CA.
In this brief overview of animal models of acute lung injury, we will begin by addressing the main features of human lung injury that are being modeled experimentally, then we will discuss the main features of some common models of ALI, followed by a discussion on specific model characteristics that may be important when deciding which model to choose, a brief technical description of key models, and end with a note on important information that should be included when reporting studies using animal models of lung injury.
What is it that is being modeled?
The key pathological features of human ALI/ARDS are a severe neutrophilic alveolitis with deposition of hyaline membrane and formation of microthrombi (Figure 1). It is important to emphasize what each of the components of the ALI/ARDS pathologic triad indicates:
- A neutrophilic alveolitis, indicating the presence of an inflammatory response in the alveoli.
- Deposition of hyaline membranes, indicating that serum proteins have entered and precipitated in the airspaces, due to disruption of the alveolar/capillary barrier, and
- Formation of microthrombi, indicating the presence of endothelial injury and intraluminal activation of the coagulation cascade.
Ideally, an “animal model of ALI/ARDS” should be a model in which this pathologic triad is reproduced, although the absence of one feature does not mean it is not a form of acute lung injury.
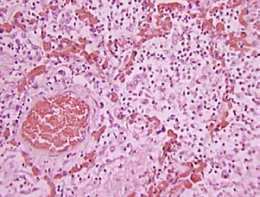
Figure 1. Human ARDS
Note the severe intra-alveolar infiltrates, deposition of hyaline membranes, vascular congestion and microthrombi.
Features of common models of acute lung injury.
Many different modeling strategies have been developed in an attempt to reproduce the features of human ALI/ARDS in animals. From a practical standpoint, there are four general types of model systems:
- Models in which the lung is injured directly by a noxious stimulus. These include the intratracheal or intranasal administration of bacteria or bacterial products such as LPS; the administration of an acid such as HCl or of gastric particulates to reproduce aspiration; the administration of high inspired fractions of oxygen; depletion of surfactant by serial lavage with 0.9% NaCl, the induction of ischemia/reperfusion by clamping the hilum, or the exposure to mechanical stretch using mechanical ventilation with high tidal volumes.
- Models in which the lung is injured indirectly. This category includes models based on reproducing sepsis, such as cecal ligation and puncture (CLP), the administration of intravenous bacteria or LPS, and mesenteric ischemia/reperfusion. This category also includes the oleic acid model, with attempts to reproduce the release of oleic acid from bone marrow in patients with multiple bone fractures.
- Combination models. To better reproduce human ALI/ARDS, different injury strategies have been combined; most commonly these include saline lavage followed by mechanical ventilation, or CLP followed by hemorrhage.
The main features of some of these models are listed in table 1
Model | Systemic features | Lung Features | |
---|---|---|---|
Direct lung injury | LPS (IT) | Mild hypotension | Acute: Patchy intra-alveolar PMN infiltrates and mild changes in epithelial permeability Repair phase: Heals without fibrosis. |
Acid Aspiration | Pulmonary hypertension | Acute phase: areas of necrosis, acute neutrophilic inflammation, hemorrhage and intra-alveolar and interstitial edema Repair phase: Heals with fibrosis |
|
Hyperoxia | No hypotension | Acute phase: Vascular congestion, alveolar exudates, PMN accumulation in vessels and interstitium Repair phase: Proliferation of type 2 cells, endothelial cells and fibroblasts, heals with areas of scarring. |
|
Surfactant depletion (saline lavage) | No hypotension | Minimal tissue injury unless followed by a second hit (such as high-volume mechanical ventilation) | |
Lung ischemia reperfusion | Acute phase: increased pulmonary vascular permeability and edema, PMN infiltration, and sometimes hemorrhage. Repair phase: Unclear (model is usually terminal). |
||
Indirect lung injury | Mesenteric ischemia reperfusion | Hypotension Metabolic Acidosis | Acute phase: Intra-alveolar proteinaceous exudates and PMN sequestration in the vasculature. |
LPS I.V. | Myocardial depression, hypotension. | Acute phase: PMN accumulation in capillaries and insterstitium but minimal intra-alveolar infiltrates, Mild changes in epithelial permeability Repair phase: Heals without fibrosis. |
|
Intravenous bacteria | Hypotension Pulmonary hypertension Decrease in CO | Acute: Interstitial edema, intravascular congestion, increased sequestration of PMN in alveolar capillaries. Minimal epithelial damage, intra-alveolar PMN infiltrates, or protein deposition in the airspaces. | |
Intrapulmonary bacteria | Hypotension | Intra-alveolar neutrophilic infiltrates, protein deposition in the airspaces. | |
Cecal ligation and puncture | Hypotension Increase in CO ulmonary hypertension | Acute: Increased epithelial permeability, PMN accumulation in the interstitium and alveolar spaces. | |
Oleic Acid (IV) | Myocardial depression, hypotension, pulmonary hypertension. | Acute phase: patchy areas of alveolar hemorrhage, intravascular thrombosis, PMN infiltration and protein-rich pulmonary edema Repair phase: Type II cell proliferation, heals without fibrosis. |
|
Combination models | Saline + MV | Minimal changes | Acute phase: Increased protein permeability, PMN infiltration into the airspaces and interstitium, hyaline membrane formation. |
CLP + hemorrhage | Hypotension | Acute phase: Interstitial edema, intra-alveolar hemorrhage, and increase in intravascular and interstitial neutrophils. |
Table 1 highlights three important points. The first is that no single model truly reproduces the pathologic findings of human ALI/ARDS that were described above. Even when all three elements of the “ALI triad” are present, they are usually milder than what is seen in humans with ARDS. The second is that models differ in the impact that they have in the individual elements of the ALI triad. For example, in some models the neutrophilic alveolitis is more marked (eg, LPS instillation into the lungs); whereas in other models the main feature is increase in intra-alveolar proteinaceous material (eg, ischemia reperfusion of the lungs). The third point is that models of sepsis are usually associated with increased deposition of neutrophils in the pulmonary vasculature and mild increases in intra-alveolar protein content, but there is less intra-alveolar infiltration of PMN or protein deposition. In other words, the lung injury that results from sepsis models is localized primarily to the vascular and interstitial compartments of the lungs, with little involvement of the alveolar spaces. The different histological characteristics of the lung injury that may be associated with selected individual models are illustrated in figures 2-9.
![]() Figure 2. Normal mouse lung |
![]() Note the predominance of neutrophilic intra-alveolar infiltrates with minimal deposition of hyaline membranes. |
![]() Note: mild intra-alveolar infiltrates, intra-alveolar deposition of protein and intravascular protein. Also note that the alveolar walls remain thin. |
![]() Zarbock et al. Note: Marked deposition of hyaline material in the alveolar spaces, abscence of intra-alveolar neutrophic infiltrates. |
![]() Perkowski et al. Balb/c mice exposed to > 95% O2 for 72 hr. Note: hemorrhagic congestion and mild intra-alveolar deposition of protiens |
![]() (Mura et al) Diffuse increase of interstitial cellularity, with both mononuclear cells and neutrophil infiltration, interstitial edema, and vascular congestion |
![]() (Imay et al J Appl Physiol 2001) Note increased celularity and areas of hemorrhage. Rabbits were lavaged with 30 cc/kg of 0.9% NaCL, then ventilated with TV = 5-6 ml/kg, PEEP = 8-10 cmH20 |
![]() (Lomas-Neira, JLB 2005) The mice were exposed to hemoorhagic shock for 90 minutes, and 24 hr later peritonitis was induced by CLP. Note the presence of interstitial edema, intra-alveolar hemorrhage, increase in intravascular and interstitial neutrophils. |
Choosing a model.
As explained above, no single animal model reproduces all the histopathological elements of ALI/ARDS satisfactorily. Thus, when choosing an animal model of ALI/ARDS, it is important to consider the key features of ALI/ARDS that will be tested by the hypothesis of the study, and then choose a model that reproduces those features. For example, an investigator interested in the mechanisms controlling neutrophil influx into the lungs will want to choose a model characterized by primarily by alveolar neutrophilia, such as LPS instillation. In contrast, an investigator interested in studying mechanisms of epithelial injury may prefer to choose a model with marked development of hyaline membranes, such as ischemia reperfusion of the lung, or acid instillation.
Model | Advantages | Disadvantages |
---|---|---|
Oleic Acid I.V. | Very reproducible Reproduces ALI triad but in milder form Reproduces repair phase | Must be given IV Mechanistic relevance unclear |
LPS I.V. | Good model of sepsis | Lung injury does not mimic human ALI/ARDS (minimal intra-alveolar neutrophilic infiltrates and protein-rich alveolar edema) |
LPS I.T. | Good model of neutrophil recruitment | Fewer changes to the epithelial barrier |
Acid aspiration | Good model of epithelial barrier disruption | Neutrophilic response is less marked than in human ALI/ARDS |
Hyperoxia | Good model of hemorrhagic injury | Less intra-alveolar neutrophilic infiltrates than in human ARDS |
Surfactant depletion | Increases lung injury induced by other agents (specially mechanical ventilation) | Little tissue injury by itself. |
Lung ischemia reperfusion | Reproduces ALI triad | Technically complex model. Repair phase difficult to study. |
Mesenteric ischemia reperfusion CLP IV LPS | Good models of sepsis | Injury is localized primarily to the vascular and interstitial compartments of the lung. |
Other key variables that should be considered include the choice of animal species. Mouse models have the advantage that many reagents are available, and there are many genetically modified strains that allow mechanistic studies. However, it is difficult (not impossible) to do physiologic measurements due to the small size. Another problem is that mice differ in some important ways from humans; for example, mice lack IL-8, which is one of the most important neutrophil chemoattractants in humans. Larger animals such as pigs and rabbits have IL-8, and are ideal for complex physiologic measurements, but they are expensive and require large volume of reagents.
Some specific models of ALI/ARDS
LPS. Lipopolysaccharide (LPS) is a glycolipid present in the outer membrane of gram-negative bacteria that is composed of a polar lipid head group (lipid A) and a chain of repeating disaccharides (1). Most of the biological effects of LPS are reproduced by lipid A (2), although the presence or absence of the repeating oligosaccharide O antigen influences the magnitude of the response (3, 4). In serum, LPS binds to a specific lipopolysaccharide binding protein (LBP) (5, 6). The LPS: LBP complex activates the CD14/TLR-4 receptor complex on monocytes, macrophages and other cells, triggering the production of inflammatory mediators (7-9). LPS is an important mediator of sepsis in response to gram-negative bacteria, and systemic administration of LPS was one of the earliest approaches used to model the consequences of bacterial sepsis.
LPS can be administered to the lungs in aerosol form, by intranasal deposition, or by direct intratracheal administration via a tracheostomy or an endotracheal catheter. The optimal dose of LPS depends on the species and strain and should be determined individually prior to a study. In addition, the lung response to LPS also depends on the type of LPS used. LPS from bacteria forming smooth colonies have the O-chain, consisting of variable numbers of repeating disaccharides, and are less pyrogenic. LPS produced by bacteria growing in rough-colonies lacks the O-chain and tend to be more pyrogenic (10). LPS preparations often contain contaminants, such as bacterial lipoproteins, and these may influence the biological effects of LPS (9).
In general, the administration of LPS is followed by changes in PMN deformability and the entrapment of PMN in the pulmonary capillaries with subsequent migration into the airspaces. PMN entrapment occurs prior to epithelial permeability changes or disruption of the alveolar / epithelial barrier (11-14). The injury is enhanced by administering first a local (intratracheal) dose of LPS, followed by a systemic (intravenous) administration. In contrast to the mild injury described in the previous paragraphs, this sequential administration of LPS results in intra-alveolar PMN infiltration, which peaks at 1-2 hr, and is followed by increased lung permeability, which peaks at 12 hr. Histopathologically the injury is characterized by diffuse interstitial edema; and alveolar exudate formation (15).
Acid Aspiration
The development of acute respiratory failure following aspiration of gastric contents was first described in 1946 by Mendelson (16) and aspiration of gastric contents is now recognized as an important risk factor for ARDS (17-19). Because one of the main characteristics of gastric contents is a low pH, HCl has been used to induce lung injury in animals. In addition to low pH, several other products such as high osmolarity, food remnants and bacterial products are present in aspirated oropharyngeal and gastric contents, and all these may have a role in the pathogenesis of aspiration-induced lung injury in humans.
Acid injury is produced by instilling HCl directly into the trachea or bronchi while the animal is mechanically ventilated. Although HCl is rapidly neutralized in the lungs, the concentration of HCl affects the severity of injury. Most studies use 0.1 N HCl, although higher concentrations (up to 0.5N) have been used. Because this results in a pH that is generally lower than that of gastric juice (most ICU patients have gastric juice pH in the range of 3.0 to 4.0 (20)), an alternative method is to use 0.3% NaCl titrated with HCl to a pH of 1.2-1.5. This results in a solution with pH and osmolarity approaching that of gastric juice.
The administration of HCl into the airway is characterized by an immediate increase in airway resistance and peak pressures, followed by increases in pulmonary artery pressure, pulmonary vascular resistance, shunt fraction and dead space. The pulmonary capillary wedge pressure and the heart rate remain unchanged, whereas the cardiac output and mean arterial pressure either remain unchanged or decrease. The main systemic consequences are neutropenia and thrombocytopenia. The lung injury is characterized physiologically by a fall in lung compliance and lung volumes (eg, functional residual capacity, FRC). The lung injury is characterized histologically by areas of necrosis, acute neutrophilic inflammation, hemorrhage and intra-alveolar and interstitial edema. The acute inflammatory response is followed one week after instillation by a fibrotic response (21).
Cecal ligation and puncture
In cecal ligation and puncture (CLP), the cecum is ligated and punctured 3 to 5 times with a needle. The abdominal incision is closed and the animal is allowed to recover. This model results in peritonitis with systemic manifestations. The severity of the injury is dependent on the number of holes in the cecum, and on the size of the needle used to make the holes (22). In general, animals become progressively leukopenic (23), but in contrast to LPS and live bacteria models, which are usually associated with a decrease in cardiac output, CLP is followed by increases in cardiac output and relatively preserved blood pressure. Pulmonary hypertension develops within 24 to 30 hours of surgery (24, 25). Blood cultures are usually positive for multiple organisms, the most common being enteric gram negative rods such as Serratia, Enterobacter and Bacteroides species (24). Animals develop hypoxemia (25) and the lungs show increased permeability, alveolar wall thickening and neutrophil accumulation in the interstitium (23, 26) and the alveolar spaces (27), within 18 to 72 hours. Mortality is high, ranging from 25% at 18 hours (27) to 70 to 90% in rats within 30 hours of the operation (23).
CLP results in mild lung injury similar to ALI/ARDS, although with less impressive intra-alveolar inflammation and hyaline membrane formation. It is probably the single best animal model of sepsis and organ injury, but the main disadvantage is the requirement for major surgery. In addition, the actual bacterial inoculum in CLP is unknown and the model could be affected by differences in colonic flora among animals, resulting in significant variability.
Reporting lung injury in manuscripts.
It is important to report measurements that provide evidence that lung injury indeed occurred in a given model. The simple elevation of the concentration of cytokines or other molecules does not provide full evidence of lung injury because these changes can occur in the absence of significant histopathological changes. Physiologic measurements alone (hypoxemia, changes in compliance) are not adequate indicators of ALI because they may be caused by conditions such as cardiogenic pulmonary edema in which no injury has occurred to the lungs. The best evidence of whether ALI has occurred in an animal is provided by a measurement of the cellular response (such as neutrophil counts in the BAL), a measurement of the integrity of the alveolar capillary barrier (such as movement of high molecular weight proteins from the serum into the airspaces) and histological images showing the lung injury.
Summary
In summary, there are many experimental models of acute lung injury, but none of them completely reproduces all the features of human ALI/ARDS. In the human disease, alveolar neutrophilia (indicating inflammation), hyaline membrane deposition (indicating disruption of the alveolar/capillary barrier) and microthrombi (indicating endothelial injury) all feature prominently. In contrast, in most experimental models of ALI only one feature predominates: alveolar neutrophilia in LPS instillation, epithelial injury in acid aspiration, etc. Therefore, no single model is the “best” model of lung injury, and instead the best model will be that which best reproduces the features that will be tested by the investigator’s hypothesis.
References.
- Raetz CR, RJ Ulevitch, SD Wright, CH Sibley, A Ding, CF Nathan. Gram-negative endotoxin: an extraordinary lipid with profound effects on eukaryotic signal transduction. Faseb J 1991;5:2652-60.
- Schromm AB, K Brandenburg, H Loppnow, AP Moran, MH Koch, ET Rietschel, U Seydel. Biological activities of lipopolysaccharides are determined by the shape of their lipid A portion. Eur J Biochem 2000;267:2008-13.
- Feist W, AJ Ulmer, J Musehold, H Brade, S Kusumoto, HD Flad. Induction of tumor necrosis factor-alpha release by lipopolysaccharide and defined lipopolysaccharide partial structures. Immunobiology 1989;179:293-307.
- Kelly NM, L Young, AS Cross. Differential induction of tumor necrosis factor by bacteria expressing rough and smooth lipopolysaccharide phenotypes. Infect Immun 1991;59:4491-6.
- Martin TR, JC Mathison, PS Tobias, DJ Leturcq, AM Moriarty, RJ Maunder, RJ Ulevitch. Lipopolysaccharide binding protein enhances the responsiveness of alveolar macrophages to bacterial lipopolysaccharide. Implications for cytokine production in normal and injured lungs. J Clin Invest 1992;90:2209-19.
- Tobias PS, K Soldau, RJ Ulevitch. Isolation of a lipopolysaccharide-binding acute phase reactant from rabbit serum. J Exp Med 1986;164:777-93.
- Wright SD, RA Ramos, PS Tobias, RJ Ulevitch, JC Mathison. CD14, a receptor for complexes of lipopolysaccharide (LPS) and LPS binding protein [see comments]. Science 1990;249:1431-3.
- Yang RB, MR Mark, A Gray, A Huang, MH Xie, M Zhang, A Goddard, WI Wood, AL Gurney, PJ Godowski. Toll-like receptor-2 mediates lipopolysaccharide-induced cellular signalling [see comments]. Nature 1998;395:284-8.
- Tapping RI, S Akashi, K Miyake, PJ Godowski, PS Tobias. Toll-Like Receptor 4, But Not Toll-Like Receptor 2, Is a Signaling Receptor for Escherichia and Salmonella Lipopolysaccharides. J Immunol 2000;165:5780-5787.
- Komuro T, C Yomota, T Kimura, C Galanos. Comparison of R- and S-form lipopolysaccharides fractionated from Escherichia coli UKT-B lipopolysaccharide in pyrogen and Limulus tests. FEMS Microbiol Lett 1989;51:79-83.
- Wiggs BR, D English, WM Quinlan, NA Doyle, JC Hogg, CM Doerschuk. Contributions of capillary pathway size and neutrophil deformability to neutrophil transit through rabbit lungs. J Appl Physiol 1994;77:463-70.
- Azghani AO, EJ Miller, BT Peterson. Virulence factors from Pseudomonas aeruginosa increase lung epithelial permeability. Lung 2000;178:261-9.
- Conroy DM, JN Francischi, P Sirois. Effect of tumor necrosis factor receptor binding protein on cell infiltration induced by lipopolysaccharide and Sephadex beads in guinea pig lung. Inflammation 1995;19:233-243.
- Wiener-Kronish JP, KH Albertine, MA Matthay. Differential responses of the endothelial and epithelial barriers of the lung in sheep to Escherichia coli endotoxin. J Clin Invest 1991;88:864-75.
- Imamura S, A Matsukawa, S Ohkawara, M Kagayama, M Yoshinaga. Involvement of tumor necrosis factor-alpha, interleukin-1 beta, interleukin-8, and interleukin-1 receptor antagonist in acute lung injury caused by local Shwartzman reaction. Pathol Int 1997;47:16-24.
- Mendelson CL. The aspiration of stomach contents into the lungs during obstetric anesthesia. Am J Obstet Gynecol 1946;52:191.
- Pepe PE, RT Potkin, DH Reus, LD Hudson, CJ Carrico. Clinical predictors of the adult respiratory distress syndrome. Am J Surg 1982;144:124-30.
- Hudson LD, JA Milberg, D Anardi, RJ Maunder. Clinical risks for development of the acute respiratory distress syndrome. Am J Respir Crit Care Med 1995;151:293-301.
- Doyle RL, N Szaflarski, GW Modin, JP Wiener-Kronish, MA Matthay. Identification of patients with acute lung injury. Predictors of mortality. Am J Respir Crit Care Med 1995;152:1818-24.
- Bonten MJ, CA Gaillard, S van der Geest, FH van Tiel, AJ Beysens, HG Smeets, EE Stobberingh. The role of intragastric acidity and stress ulcus prophylaxis on colonization and infection in mechanically ventilated ICU patients. A stratified, randomized, double-blind study of sucralfate versus antacids. Am J Respir Crit Care Med 1995;152:1825-34.
- Yano T, RR Deterding, WS Simonet, JM Shannon, RJ Mason. Keratinocyte growth factor reduces lung damage due to acid instillation in rats. Am J Respir Cell Mol Biol 1996;15:433-42.
- Walley KR, NW Lukacs, TJ Standiford, RM Strieter, SL Kunkel. Balance of inflammatory cytokines related to severity and mortality of murine sepsis. Infect Immun 1996;64:4733-8.
- Goya T, M Abe, H Shimura, M Torisu. Characteristics of alveolar macrophages in experimental septic lung. J Leukoc Biol 1992;52:236-43.
- Gnidec AG, WJ Sibbald, H Cheung, A Craig. Ibuprofen reduces the progression of permeability edema in an animal model of hyperdynamic sepsis. J Appl Physiol 1988;65:1024-1032.
- Lewis JF, R Veldhuizen, F Possmayer, W Sibbald, J Whitsett, R Qanbar, L McCaig. Altered alveolar surfactant is an early marker of acute lung injury in septic adult sheep. Am J Respir Crit Care Med 1994;150:123-30.
- Olson LM, GS Moss, O Baukus, TK Das Gupta. The role of C5 in septic lung injury. Ann Surg 1985;202:771-776.
- Villar J, SP Ribeiro, JB Mullen, M Kuliszewski, M Post, AS Slutsky. Induction of the heat shock response reduces mortality rate and organ damage in a sepsis-induced acute lung injury model [see comments]. Crit Care Med 1994;22:914-21.